Thermohaline Processes - Water Mass Formation - The Seasonal Thermocline...
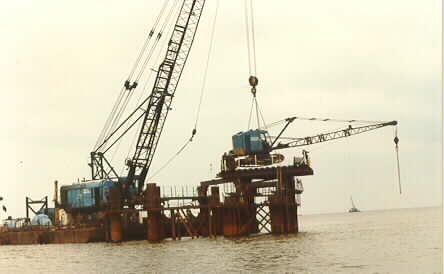
In most ocean regions the wind-driven circulation, which was the focus of discussion so far, does not reach below the upper
kilometre of the ocean. Water renewal below that depth is achieved by currents which are driven by density differences
produced by temperature (thermal) and salinity (haline) effects. The associated circulation is therefore referred to as
the thermohaline circulation. Since these movements are mostly very sluggish, it is often impractible to use current meters
to measure them directly; they are usually deduced from the distribution of water properties and the application of geostrophy.
The driving force for the thermohaline circulation is water mass formation. Water masses with well-defined temperature and
salinity characteristics are created by surface processes in specific locations; they then sink and mix slowly with other
water masses as they move along. The two main processes of water mass formation are deep convection and subduction. Both
are linked to the dynamics of the mixed layer at the surface of the ocean; so it is necessary to discuss thermohaline
aspects of the upper ocean first.
Oceanographers refer to the surface layer with uniform hydrographic properties as the surface mixed layer. This layer is an
essential element of heat and freshwater transfer between the atmosphere and the ocean. It usually occupies the uppermost
50 - 150 m or so but can reach much deeper in winter when cooling at the sea surface produces convective overturning of
water, releasing heat stored in the ocean to the atmosphere. During spring and summer the mixed layer absorbs heat,
moderating the earth's seasonal temperature extremes by storing heat until the following autumn and winter, and the deep
mixed layer from the previous winter is covered by a shallow layer of warm, light water. During this time mixing is achieved
by the action of wind waves, which cannot reach much deeper than a few tens of meters. Below the layer of active mixing is
a zone of rapid transition, where (in most situations) temperature decreases rapidly with depth. This transition layer is
called the seasonal thermocline. Being the bottom of the surface mixed layer, it is shallow in spring and summer, deep in
autumn, and disappears in winter, when heat loss at the surface produces instability and the resulting convection mixes the
water column to greater depth (
). In the tropics winter cooling is not strong enough to destroy the seasonal thermocline, and a shallow feature sometimes
called the tropical thermocline is maintained throughout the year.
The depth range from below the seasonal thermocline to about 1,000 m is known as the permanent or oceanic thermocline. It
is the transition zone from the warm waters of the surface layer to the cold waters of great oceanic depth The temperature
at the upper limit of the permanent thermocline depends on latitude, reaching from well above 20°C in the tropics to just
above 15°C in temperate regions; at the lower limit temperatures are rather uniform around 4 - 6°C depending on the
particular ocean. Below the surface layer which is in permanent contact with the atmosphere, temperature and salinity are
conservative properties, ie they can only be changed by mixing and advection. All other properties of sea water such as
oxygen, nutrients etc. are affected by biological and chemical processes and therefore non-conservative. Water masses can
therefore be identified by their temperature-salinity (T-S) combinations (
). Water mass formation by deep convection occurs in regions with little density stratification (ie mostly in polar and
subpolar regions). When the water in the mixed layer gets denser than the water below, it sinks to great depth, in some
regions to the ocean floor. The density increase can be achieved by cooling or an increase in salinity (either through
evaporation or through brine concentration during freezing) or both. Water mass formation by subduction occurs mainly in
the subtropics. Water from the bottom of the mixed layer is pumped downward through a convergence in the Ekman transport
and sinks slowly along surfaces of constant density (
).
gives a summary of water masses in the world ocean. Antarctic Bottom Water is formed mainly in the Weddell and Ross Seas by
deep convection and fills all ocean basins below 4,000 m depth; in the Pacific and Indian Oceans it is mixed with North Atlantic
Deep Water, the mixture being known as Circumpolar Water. North Atlantic Deep Water is the product of a process that involves
deep convection in the Arctic Ocean, the Greenland Sea and the Labrador Sea. Most Antarctic Intermediate Water is formed by
deep convection east of southern Chile and west of southern Argentina and spreads into all oceans with the Circumpolar
Current. Intermediate Water in the northern hemipshere may be formed by convection or subduction. Central Water, the water
of the permanent thermocline, is formed by subduction in the subtropics. Mediterranean and Red Sea waters are intrusions of
high temperature, high salinity waters from two mediterranean seas (see the discussion of mediterranean seas below).
It is worth stressing that the picture developed here is of a very schematic nature. The real ocean is a fluid in turbulent
motion full of eddies, fronts and other instabilities. It should also be kept in mind that significant zonal (east-west
directed) movement occurs in every ocean basin and that the distribution cannot depict the variations that occur from the
eastern to the western coasts. However, as a summary of the principal features of the water masses in the world ocean it is
correct and adequate. A summary of the TS characteristics of all water masses is given in
. Antarctic Bottom Water is represented by a single TS point (in the white region of the salinity scale). Antarctic Intermediate
Water also has its own TS point but is usually only seen as a salinity minimum in the TS-curve; the minimum is slowly eroded
by mixing as the Intermediate Water progresses northward (
). The Central Waters are represented by TS curves rather than points. A complete description of water mass movement
requires horizontal property distributions as well as vertical sections and TS-diagrams. It is then seen that the path
of Antarctic Bottom Water in particular is strongly affected by the topography. For example, the deep basins of the eastern
Atlantic Ocean are separated from the Southern Ocean by a sill and cannot be reached by Antarctic Bottom Water directly.
They are filled through a gap in the Mid-Atlantic Ridge near the equator known as the Romanche Fracture Zone; in other
words, flow of Antarctic Bottom Water in the eastern South Atlantic Ocean is southward, from the equator toward the pole.
In the Pacific Ocean, input is mainly along 170°W (east of New Zealand), followed by spreading east and westward in the
northern hemisphere; recirculation into the southern hemisphere occurs in the east. Input into the Indian Ocean is from
the west, and in smaller quantities from the east.
Circulation in Mediterranean Seas...
Mediterranean Seas are large bodies of water characterized by very restricted water exchange with the major ocean basins.
This results in different hydodynamics and sets them apart from the remainder of the world ocean. While the circulation in
most of the world ocean is dominated by wind-driven currents, the circulation in mediterranean seas is determined by
thermohaline processes. Two basic types of circulation can be distinguished, the concentration basin and the dilution
basin. Concentration basins occur where evaporation in the region exceeds precipitation; such mediterranean seas are
therefore also sometimes called arid mediterranean seas. Examples are the (Eurafrican) Mediterranean Sea, the Red Sea and
the Persian Gulf. Dilution basins occur when precipitation and river input exceed evaporation; such basins are therefore
also known as humid mediterranean seas. Examples are the Black Sea, the Baltic Sea and the Australasian Mediterranean
Sea (the seas of the Indonesian archipelago).
The circulation in mediterranean seas and their water exchange with the remainder of the world ocean differs strikingly
between the two types (
). In concentration basins (
), evaporation increases the salinity of the surface waters, raising their density and producing convection. Deep water
renewal is therefore a nearly continuous process, and the waters of the basin are well ventilated (have relatively high
oxygen content) at all depth. In dilution basins, the freshening of the surface waters resulting from excess rain and
freshwater input from rivers reduces the density of the surface layer. This prevents the freshened water to reach the
deeper layers. The result is the establishment of a fresh upper layer and a strong halocline. Water below the halocline
is renewed only very slowly through mixing across the halocline and inflow of oceanic water through the connecting strait.
Oxygen at depth is consumed by remineralisation of nutrients. Oxygen content is therefore very low when active ventilation
is inhibited through the stable halocline (
). If the basin is large and the exchange with the open ocean very restricted, oxygen levels at depth can fall to zero,
preventing the existence of higher marine life. Such conditions are occasionally found in some basins of the Baltic Sea.
The Black Sea, which is more than 1,500 m deep, is devoid of oxygen below 150 m depth.
The Ocean and Climate...
Ocean and atmosphere form a coupled system. The coupling occurs through exchange processes at the interface (the sea
surface). These exchange processes determine the energy and mass budgets of the ocean. Quantities exchanged between the
ocean and the atmosphere are ; (1) In the energy budget : (a)radiative energy (including heat), (b) momentum ; (2) In the
mass budget : (a) freshwater, through evaporation / condensation and precipitation / river run-off, (b) minerals, (c) gases.
Gases absorb radiative energy selectively. Some gases are transparent to the short wave radiation emitted by the sun but
highly absorbent at the low (infra red) wave lengths at which the earth emits radiative energy into space. High concentration
of these gases in the atmosphere leads to a trapping of radiative energy in the atmosphere which manifests itself as an
increase in the atmospheric temperature. These gases are therefore known as greenhouse gases. Carbon dioxide is a particularly
important greenhouse gas. The role of the ocean in the earth's climate and its capability of storing carbon dioxide (CO2)
is discussed in the following text. Some annotations, which are not part of the original text, were added to make the text
more accessible to first year students; these annotations are in orange.
Begin of Quotation...
The ocean plays a role in the climate system which is complementary and of comparable importance to that of the atmosphere.
It stores heat and releases it later, and often in a different place. It transports heat in amounts comparable with atmospheric
transport. It both absorbs and releases carbon dioxide. It is sometimes referred to as the "flywheel of the climate system".
Like a flywheel, the ocean stores energy, in this case thermal energy, when it is in large supply during the day or summer,
and releases it when the energy supply is reduced or reversed during night or winter. When it is heated the ocean responds
by storing some of the heat and by increased evaporation. Because the heat is mixed down for some metres by the wind,
temperature rises much less than it does on dry land under the same heating conditions. The evaporation has profound effects
on the atmosphere and on climate. Water vapour released into the atmosphere importantly increases the greenhouse effect in
the atmosphere. When it recondenses, the resulting heating of the air is a major source of energy for atmospheric motion.
When the ocean is cooled, it responds by generating vertical convective motions, which resupply heat to the surface. (This
occurs because continuity of mass requires that cold water sinking from the surface is replaced by water from below. This
water is - slightly - warmer than the sinking water and thus represents a supply of heat.) Thus the temperature fall is
much less than over land under the same cooling conditions.
The overall result is that for the two thirds of the earth's surface covered by ice-free ocean, the temperature over the
whole ocean ranges only from -2°C (the freezing point of salt water) to 30°C, and at any one place by hardly more than 1°C
during the course of a day and 10°C during the course of a year. This range might be compared with that over dry mid continental
areas, where the variation from place to place can be about 100°C, and during the course of the year in particular places
about 80°C. Further, the relatively slow response of the ocean to heating and cooling results in the oceanic annual cycle
being retarded relative to that in continental regions. (Much more energy is required to change the temperature of water
than the temperature of air, so the ocean takes longer to heat up or cool down. As a result the ocean is still warming up
in late summer when the air is still warmer then the water but already cooling, and it is still cooling down in late winter
when the air is still cooler than the water but the atmosphere is already warming.).
Such effects would be experienced even if the ocean were little more than a deep swamp. However, the ocean moves. In moving,
it redistributes heat (and salt) in ways that are of central importance in determining the details of the earth's climate.
The North Atlantic provides a particularly notable example. In the tropical Atlantic, solar heating and excess evaporation
over precipitation and runoff creates an upper layer of relatively warm, saline water. Some of this water flows north,
through the passages between Iceland and Britain. On the way it gives up heat to the atmosphere, particularly in winter.
Since winds at these latitudes are generally from the west, the heat is carried over Europe, producing the mild winters which
are so characteristic of that region relative to others at similar latitudes.
So much heat is withdrawn (from the ocean and absorbed by the overlying atmosphere) that the temperature (of the water at
the ocean surface) drops close to the freezing point. This water, now in the Greenland Sea, remains relatively saline, and
the combination of low temperature and high salinity makes the water more dense than deeper water below it. Convection sets
in and the water sinks - occasionally and locally right to the bottom. There it slides under, and mixes with, other water
already close to the bottom. It spreads out and flows southward, deep and cold. This thermohaline circulation: surface warm
water flowing north, cooling, sinking and then flowing south provides an enormous northward heat flux. It amounts to 1PW
(petawatt, 1 PW = 1 billion megawatts), fully comparable with that transported poleward by the atmosphere.
Water (from all depths) is in repeated contact with the surface and comes into approximate equilibrium with atmospheric
concentrations of gases, including notably O2, CO2 and freons. The freons are inert (not influenced
by biological processes or chemical reactions; their concentration is only affected by the mixing of water), and provide a
valuable passive tracer for ocean movement. O2 and CO2, on the other hand, are strongly affected by
biological activity. The surface layers of the ocean contain planktonic plants which, in the presence of sunlight, convert
dissolved CO2 into organic carbon. The plants are eaten by animals, which are in turn consumed by other organisms.
Debris from these organisms falls out of the surface layers into the deeper water. On the way down, bacteria decompose some
of the material, releasing CO2 and absorbing O2. As a result the deeper water is enriched in CO2
and nutrients and depleted in O2.
The ocean plays a key, but frequently understated, role in determining the earth's climate. Indeed any possibility of
predicting the evolution of climate beyond a few weeks demands that ocean behaviour also be taken into account. With
respect to sensitivity to, and contribution to, long term climate change: there is every reason to believe that the ocean
is now changing, in response to climate changes over the past few hundred years (the Little Ice Age). It can be expected to
change further as anthropogenic influences (influences from human activity) become increasingly marked. The effect of the
ocean on the atmosphere could be either to moderate or to intensify these changes. It will certainly modify them. The map
shows how the ocean circulation distributes heat throughout the world's oceans.
End of Quotation...
The thermohaline circulation described above ("surface warm water flowing north, cooling, sinking and then flowing south")
has become known as the Great Ocean Conveyor Belt (
). The water that sinks in the North Atlantic Ocean (North Atlantic Deep Water) enters the Antarctic Circumpolar Current
and from there all ocean basins, where it rises slowly into the upper kilometre and returns to the North Atlantic in the
permanent thermocline. Although this is only one of the circulation paths of North Atlantic Deep Water it is the most
important one from the point of ocean/atmosphere coupling since it acts as a major sink for atmospheric greenhouse gases.
The only other region of similar importance is the Southern Ocean where Antarctic Bottom Water sinks.
Formation of North Atlantic Deep Water does not necessarily continue forever. The deep convection in the Greenland Sea
occurs in a region where cold, fresh water and warm, saline water meet (
). Convection occurs when the warm, salty water gets cold enough to sink, just before its relatively high density forces it
to slide underneath the fresh polar surface waters and continue as a subsurface current. Convection can be inhibited by
a number of processes. If the climate gets warmer, additional melting of ice will increase the volume of the Arctic outflow
of cold, fresh water and push the region where the warm, salty current is forced to underneath the fresh polar surface
waters further to the south. The warm, salty water is then insulated from atmospheric cooling and will no longer sink.
This will stop the conveyor belt. As a result, Europe will become much colder, more ice will form in the Arctic, outflow of
cold, fresh water will be reduced, and the conveyor belt will be active again. It is seen that the ocean can support two
alternate circulation systems as the two states of an oscillation system. There is geological evidence that the conveyor
belt is inactive during ice ages. The question whether and to which degree the thermohaline circulation is susceptible to
human activity is the subject of intense research in numerous institutions around the world.
El Niño and the Southern Oscillation (ENSO)...
The discussion of ocean circulation changes and ice ages above gave one example of oscillatory behaviour of the coupled
ocean/atmosphere system. Another example, on a time scale short enough to be experienced during a human life span, occurs
in the Pacific Ocean and is known as ENSO, which stands for El Niño - Southern Oscillation. The Southern Oscillation is the
term for a large-scale oscillation of air pressure observed in the tropics around the globe and particularly clearly over
the tropical Pacific Ocean, where air pressure is high in Darwin when it is low in Tahiti (or the central and eastern
Pacific Ocean in general) and vice versa.
shows the effect of the Southern Oscillation on air pressure and rainfall. It is seen that high air pressure at Darwin is
linked with high rainfall in the central Pacific. El Niño is the name for the oceanographic side of the phenomenon. One of
the richest fishing regions of the World Ocean, the South Pacific coastal upwelling region along the coast of Peru, Chile,
and Ecuador, occasionally experiences an influx of nutrient-poor, warm tropical water which suppresses the upwelling of
nutrients. The anchoveta which inhabit these waters in their millions forming the nutritional basis for a huge bird
population and the stock for an important fish meal industry, depend on the supply of nutrients to the surface layer.
They avoid the warm nutrient-poor water, which causes mass mortality amongst the birds. If the extent of the tropical
influx is very severe, mass mortality can occur among the fish as well; hydrogen sulphide from decaying fish has been known
to blacken the paint on ships in Callao harbour. When this occurs it occurs usually just before Christmas - thus the name
"El Niño" (the child) which relates the event to the birth of Christ. The high temperatures along the South American coast
last for about a year or more before conditions return to those which prevailed before the influx of tropical water.
A simplified description of the mechanism how ocean and atmosphere interact to cause an ENSO events starts from the effect
of sea surface temperature on winds.
indicates that the two convergence zones in the atmosphere coincide with regions of high sea surface temperatures. This is
because the air is heated where the water is warm; it rises, producing a convergence of the winds above the sea surface
(seen in high average cloud cover,
) - in other words, sea surface winds blow towards regions of high sea surface temperature. This results in accumulation of
warm water, which increases the heating; the air rises faster, wind speed increases - a positive feedback is established.
Suppose now that through some disturbance the region of highest temperature is shifted from the region where the ITCZ and
the SPCZ meet (point A) to a point somewhere further east (point B). Winds continue to blow towards the highest sea surface
temperature; so the winds to the west of that point will reverse their direction and change from Trades to westerlies. Again,
this pattern will be reinforced through positive feedback. The centre of rainfall is shifted from A to B; drought conditions
are observed in Australia. This is often accompanied by tropical cyclone development (
). The change from one state of the ocean/atmosphere feedback system to the other requires unstable conditions in the
atmosphere. Such conditions occur usually around May or June when the atmospheric circulation over the adjacent Indian
Ocean changes from Northeast to Southwest Monsoon. Whether an ENSO event occurs in a particular year is therefore usually
decided in May.
The westerly winds in the western equatorial Pacific trigger a solitary internal wave of large scale (several hundreds of
kilometers in length and about 400 km across) which travels eastward along the equator, advecting warm tropical water into
the South American coastal upwelling region. The detailed dynamics are complicated and involve several types of long oceanic
waves of very low frequency which take between 1 - 4 months to cross the equatorial Pacific Ocean and alter the temperature
of the upper ocean thousands of miles from where they were generated. What is important in the process is that the original
disturbance in the ocean/atmosphere system which occurred in the western Pacific Ocean early in the year produces a suppression
of coastal upwelling and an influx of tropical water along the coast of Peru, Chile and Ecuador later in the year. The suppression
of upwelling is most intense in November - December and does not disappear until well into the following year.
Waves...
Waves are periodic deformations of an interface. Surface waves in oceanography are deformations of the sea surface, i.e.
the atmosphere-ocean interface. The deformations propagate with the wave speed, while the particles describe orbital or
oscillatory motions at particle speed and remain at the same position on average. In deep water, particle paths are circles.
In shallow water, the particle paths flatten to ellipses (
). The change from deep to shallow water waves occurs
when the wavelength l becomes larger than twice the water depth h. It is therefore useful to distinguish between ;
l / 2 > h : shallow water waves or long waves
l / 2 < h : deep water waves or short waves
It is most important to note that the distinction between deep and shallow water waves has little to do with absolute water
depth but is determined by the ratio of water depth to wave length. The deep ocean can be shallow with respect to waves
provided the wave length exceeds twice the ocean depth. This is the case for example with tides.
Wave Classification...
Ocean waves can be classified in various ways. One classification uses the forces which generate the waves : (1) Tides
(astronomical forcing); they are always shallow water or long waves, (2) Meteorological forcing (wind, air pressure); sea
and swell belong to this category, (3) Earthquakes; they generate tsunamis, which are shallow water or long waves. Another
classification is based on the frequency spectrum representation of all oceanic waves and distinguishes between capillary
waves, gravity waves, long period waves, tides and transtidal waves (
). Yet another classification is based on the restoring forces responsible for returning the water particles to their
average position in the water column.
Description of Waves...
The simplest way of looking at waves is the concept of a wave as a harmonic oscillation (
). It can then be described by its ;
Parameter |
Definition |
Period |
t |
Frequency |
w = 2 p / t |
Wavelength |
l |
Wave speed |
c = l / t |
Wave height |
H = 2A (A = amplitude) |
Wave steepness |
d = H / l |
Superposition of two waves with nearly equal frequencies w1 and w2 produces wave groups or packets (
). Individual wave crests travel with phase velocity (identical to the wave speed) c; wave packets travel with group
velocity ;
cg = c - l (dc / dl)
Although wave particles remain at the same position on average, waves transport energy in packets. Energy propagates with group velocity; it can move faster or
slower than individual wave crests. This is called dispersion.
Normal Dispersion...
In normal dispersion, c increases with l, i.e. the crests of long waves travel faster than the crests of short waves. As a
consequence cg < c, i.e. energy travels slower than the wave crests. This occurs with gravity waves such as swell.
Nondispersive Waves...
Here, cg = c, i.e. all wave crests travel at the same speed, and energy propagates at the same speed.
Anomalous Dispersion...
This is found when cg > c. Capillary waves are an example. Energy propagates faster than wave crests, and short waves travel
faster than long waves. In most oceanic situations the wave steepness is very small and the wave speed is then given by ;
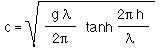
(valid for d < < 1, or l < < H)
Here, H is the wave height and h the water depth. The formula can be simplified depending on the ratio of l against h ;
Deep water wave speed (short waves)
cg = c / 2 (normal dispersion)
Shallow water wave speed (long waves)
cg = c (nondispersive waves)
As an example, consider waves produced by a distant storm. In the open ocean such waves travel as deep water waves; their
wave speed therefore depends on their wavelength l. Thus long waves travel fastest and arrive at distant locations first.
They are experienced as swell. When swell approaches a beach the water depth decreases and there comes a point when the
waves change from deep water waves to shallow water waves. As a consequence the wave speed c decreases as the depth decreases
and the waves bend inwards; the wave front becomes progressively more parallel to the beach.
Waves of Finite Amplitude...
The harmonic oscillation concept gives a good description of waves of very small steepness. On the approach of a beach, or
during the period of active formation by wind, wave steepness is not small enough and the wave profile deviates from the
harmonic profile. As the steepness increases, the wave profile becomes cusped :
Eventually, the waves break. The limiting values before breaking are 120° for the cusp angle and a steepness d = 1/8.
Cusped waves do not have closed particle paths but are associated with a net transport of water (not just energy). This
transport is called Stokes drift, after its investigator.
Short Waves (Deep Water Waves)...
Short waves in the ocean are wind generated waves. They are divided into sea and swell. Sea includes all waves generated by
the local winds, while swell refers to waves generated by distant wind fields. The effect of the wind on the state of the
sea depends on the distance over which the wind can blow without hindrance before it reaches the observation point. This
distance is known as the fetch. The presence of a coast limits the fetch for winds blowing from land to sea. In the open
ocean the fetch is usually determined by the size of the weather system that produces the wind. At any given instant in
time the sea state is never a single harmonic oscillation. Therefore ways have to be found to describe the wave conditions
in terms of measurable quantities. Two approaches are used ; (1) Determination of significant wave parameters and (2)
Statistical description (spectrum)
The combination of many measurements has resulted in wave parameter estimates for fully developed seas (
). A fully developed sea is one for which the fetch and duration are not limiting ie no further growth occurs, as momentum
and energy loss through breaking balances input from the wind. The graph shown in the figure shown above applies to situations
where waves are generated by local wind.
shows wave properties for situations where the waves are generated remotely. It assumes fully developed sea conditions in
the generation region. For many marine applications, for example the routing of ships or the design of platforms, only the
heighest waves are of interest. The quantity significant wave height has therefore been introduced. It is defined as either
H1/3 or H1/10, i.e. as the average of the 1/3 or 1/10 heighest waves over an observation period. From observations, the
largest wave height Hmax is related to the significant wave height by ;
and
Statistical Description of Waves...
The relations between largest wave heigth and significant wave height indicate that the sea state has certain statistical
properties. A statistical description is based on the representation of the wave field through the energy spectrum. For a
given frequency, wave energy is proportional to the square of the amplitude. An energy spectrum shows wave energy as a
function of wave frequency. A single harmonic wave has a 'monochromatic' spectrum ;
.
In a wind sea with random distribution of wave energy over all wave frequencies, the theoretical form of the energy spectrum
is that of a Gaussian or 'normal' distribution. Where only swell is present the energy is concentrated near the swell frequency
and the spectrum is much narrower ;
.
Statistical description assumes that waves of all frequencies and corresponding wavelengths are present. It does not attempt
to describe the form of the sea surface but concentrates on wave energy.
shows observed energy spectra for fully developed seas at various wind speeds. Note that the spectrum has a normal distribution
only for very low wind speeds; as the wind speed increases, waves of short period are still present but most of the energy is
found in longer period waves. The spectrum then drops off rapidly at longer periods; it is "skewed" towards the longer periods.
Final wave decay occurs during breaking on a beach, which occurs when the particle velocity becomes larger than the phase velocity
(wave speed). It is coupled with strong transport of energy and mass in the rip current system.